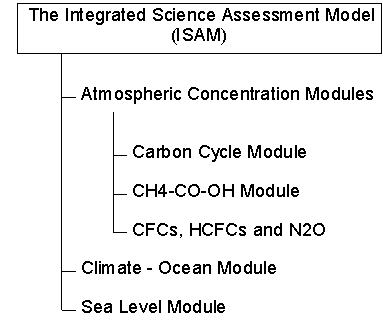
INTREGRATED ASSESSMENT MODELING (IAM)
Global climate change is perhaps the most significant environmental issue of our time. A comprehensive assessment of the scientific evidence by the Intergovernmental Panel on Climate Change (IPCC), co-sponsored by the United Nations Environment Program (UNEP) and the World Meteorological Organization (WMO) and made up of over 2000 scientific and technical experts from around the world, suggests that human activities are contributing to climate change and there has been a discernible human influence on global climate (Harvey et al., IPCC, 1996).
The human activities, most importantly the burning of fossil fuels, as well as deforestation and various agricultural and industrial practices, have led to increased atmospheric concentrations of the number of greenhouse gases, including carbon dioxide, methane, nitrous oxide, chloroflurocarbons, and ozone in the lower part of the atmosphere. The basic heat-trapping property of these greenhouse gases is essentially undisputed. Although there is considerable scientific uncertainty about exactly how and when the earth’s climate will respond to enhanced greenhouse gases in the future. The direct effects of climate change will changes in temperature, precipitation, soil moisture and sea level. Such changes could have adverse effects on ecological systems, human health and socio-economic sectors.
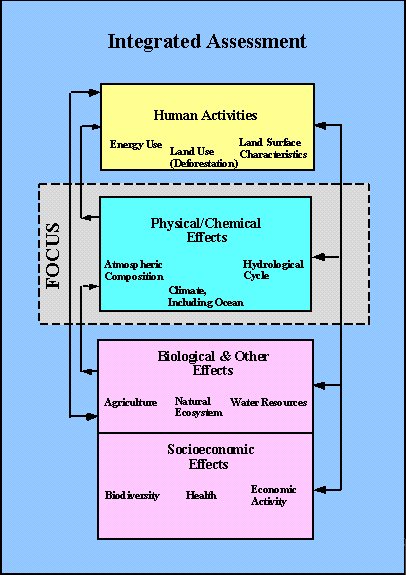 |
Figure 1: Click image to enlarge |
Thus, understanding of the relationships between human activities, potential changes to the Earth’s climate, and the resulting ecological and economic impacts and other effects on human welfare from these changes requires an interdisciplinary perspective involving the physical, biological, social and political sciences (Figure 1). In response to this need, a new paradigm has emerged - one whose main purpose is not only the acquisition of scientific knowledge, but the assimilation and communication of scientific results. Integrated Assessment Modeling (IAM) is a new important research methodology for examining the complex interactions among physical, and human systems. Rather than actually using many of the multi-dimensional and complicated expert models, IAM build on the knowledge achieved by each individual scientific discipline. The uses of such tools need to explicitly recognize and address the existence of considerable uncertainty and scientific debate surrounding climate issues. The model must be computationally efficient so that the many possibilities to be considered can be investigated on a personal computer or workstation in a matter of minutes, and must be simple and transparent, but based on solid science.
INTEGRATED SCIENCE ASSESSMENT MODEL (ISAM)
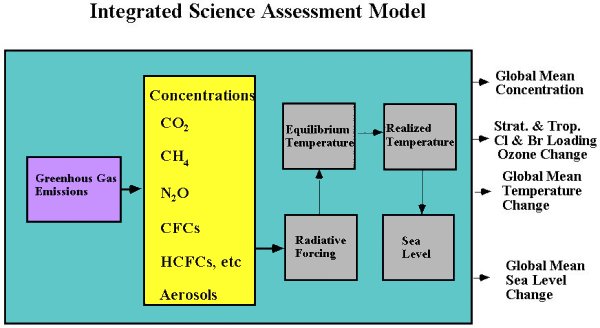 |
Figure 2: Click image to enlarge |
Our existing Integrated Science Assessment Model (ISAM) for assessment of climate change (Jain et al., 1994) consists of coupled modules for representation of the carbon cycle, effects of greenhouse gas emissions and aerosols on atmospheric composition, effects on global temperatures using an energy balance model, and processes affecting sea level change (Figure 2). This model has been used to estimate the relation between the time-dependent rate of greenhouse gas emissions and quantitative features of climate global temperature, the rate of temperature change, and sea level that are thought to be indicators of human impact on climate and ecosystems (Wigley et al., 1998). This model has also been applied to studies of Global Warming Potential (GWP, Wuebbles, et al., 1995), and the Economic-Damage Index (EDI, Hammitt et al., 1996) concepts.
CONCENTRATIONS MODULES
Carbon Cycle Model
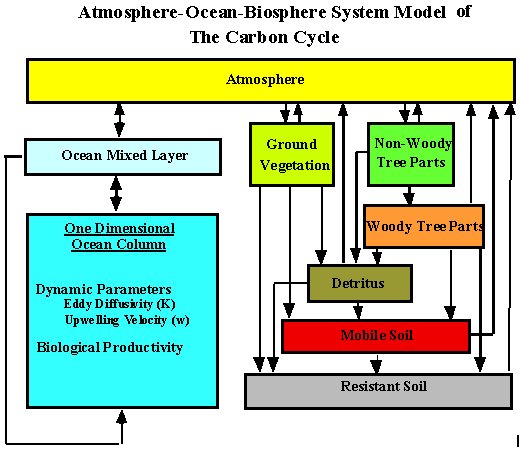 |
Figure 3: Click image to enlarge |
The concentration of CO2 is calculated by a globally averaged carbon cycle model (Figure 3), which consists of four reservoirs, namely the atmosphere, the terrestrial biosphere, the mixed ocean layer, and the deep ocean (Jain et al., 1995, 1996). The atmosphere and the mixed layers are modeled as well mixed reservoirs. Transport of total inorganic carbon in the deep ocean is modeled by a partial differential equation, spanning time and ocean depth, which accounts for vertical diffusion and upwelling. The rate of transport in the deep ocean is dependent on two parameters: eddy diffusivity (k) and upwelling velocity (w). (Jain et al. 1995) determined parameter values k = 4700 m2/yr and w = 3.5 m/yr by calibration of model results to the estimated global-mean pre-anthropogenic depth-profile of ocean 14C concentration). Water upwells through the deep ocean column to the surface ocean layer from where it is returned, through a polar sea, to the bottom of the ocean column thereby completing the thermohaline circulation. Air-sea exchange is modeled by an air sea exchange coefficient in combination with the buffer factor that summarizes the chemical re-equilibration of sea water with respect to CO2 variations. The buffer factor is calculated from the set of equations for borate, silicate, phosphate, and carbonate equilibrium chemistry and the temperature-dependent equilibrium constants. An additional source term is added to the model deep ocean to account for the oxidation of organic debris containing carbon removed in the surface ocean layer by photosynthesis and brought to the deep ocean by particulate settling.
To estimate the flux of CO2 between the terrestrial biosphere and the atmosphere, a 6 box, globally-aggregated, terrestrial-biosphere sub-model is coupled to the atmosphere box. The six boxes represent ground vegetation, non-woody tree parts, woody tree parts, detritus, mobile soil (turn-over time 75 years), resistant soil (turnover time 500 years). The mass of the carbon contained in the different terrestrial reservoirs and the rate of exchange between them have been based on the analysis by Kheshgi et al. (1996). The photosynthetic rate of carbon fixation is modeled to increase logarithmically with atmospheric CO2 concentration and is proportional to a CO2 fertilization factor. The rate of photosynthesis by terrestrial biota is thought to be stimulated by increasing atmospheric carbon dioxide concentration. The increase in the rate of photosynthesis, relative to preindustrial times, is modeled to be proportional to the logarithm of the relative increase in atmospheric CO2 concentration from its pre-industrial value of 278 ppm. The proportionality constant b, known as the CO2 fertilization factor, is chosen to be 0.42. The rate coefficients for exchange to and from terrestrial biosphere boxes are temperature dependent according to an Arrhenius law.
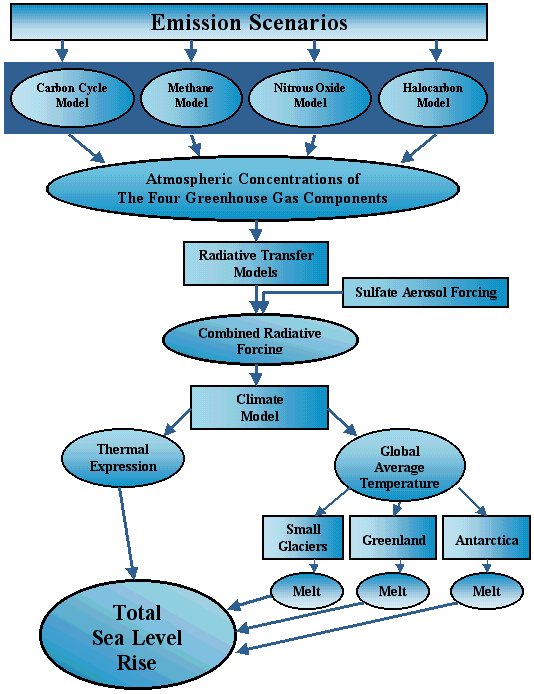 |
Figure 4: Click image to enlarge |
This model does contain temperature feedbacks on carbon cycle through the prescription of the temperature-dependent buffer factor, and exchange (respiration and photosynthesis) rates to and from the biosphere boxes which follow the "Q10 formulation" described in Kheshgi et al. (1996).
The existing carbon cycle model in ISAM was originally developed as a tool to predict the likely future changes of the atmospheric abundance of CO2 dependent on the use of fossil fuels, deforestation and expansion of agriculture land. This schematic model for the carbon cycle is constructed to be consistent with current understanding of the global carbon cycle. Testing of the capability of the model to represent this understanding has, in part, been based on the analysis of tracer records such as those for 13C and 14C (Figure 4). For the last two IPCC assessments, our carbon cycle model has also been used to assess the future scenarios (Schimel et al., 1995, 1996, 1997).
CH4-CO-OH Cycle Module
The methane concentration in the atmosphere is calculated by simulating the main atmospheric chemical processes influencing the global concentrations of CH4, CO, and OH, using the global CH4-CO-OH cycle module (Figure 5). The removal rates of CH4 and CO are determined by accounting for the uptake by soils, transport to the stratosphere, and oxidation by OH radicals. We assume the uptake velocity by soils and the transport velocity to the stratosphere to be time-independent, although there is evidence that these values change with time.
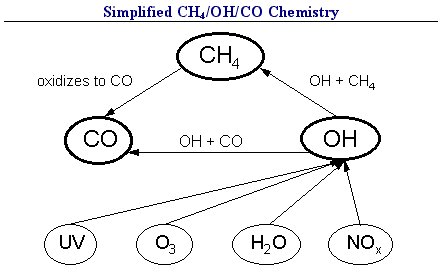 |
Figure 5: Click image to enlarge |
Reaction with the OH radical is responsible for up to 90% of the tropospheric CH4 sink, making the tropospheric concentrations of OH and CH4 the most important factors influencing the rate at which CH4 is destroyed. OH concentration is primarily determined by the concentrations of CH4, CO, NOx, non-methane hydrocarbons (NMHCs), tropospheric ozone, and water vapor. With sharp spatial and temporal gradients, an observation-based estimate of the global concentration of OH is difficult to make, as are the rates of OH production and destruction which depend non-linearly on other atmospheric constituents. In attempts to adequately represent all significant chemical and dynamic processes determining local OH concentrations, detailed two- or three-dimensional chemical transport models of the atmosphere have been used. However, to incorporate OH chemistry into methane modeling for scenario analysis, simplified schemes are used which reproduce the important influences on OH while remaining straightforward and computationally feasible. In ISAM, the concentration of OH is determined by the photochemical balance between the total tropospheric production of OH and the loss rate due to reaction with CH4, CO and NMHCs [Jain et al., 1994]. The loss rate of OH is determined by reactions between OH and CH4, CO and NMHCs with reaction rates. The production rate of OH is based on a correlation giving global OH production rate as a function of NOx emissions and CH4 concentration based on results of a well established 2-D (latitude and altitude) chemical-radiative transport model of the global atmosphere (Wuebbles et al., 1991, 1998). The 2-D model used has a full representation of NOx, HOx, CH4, O3, and CO chemistry relevant to tropospheric and stratospheric chemistry. The OH production rate correlation used in ISAM was derived from the following results of this 2-D model: a 110-year simulation of the Intergovernmental Panel on Climate Change (IPCC) scenario IS92a and perturbations from 1990 atmospheric conditions.
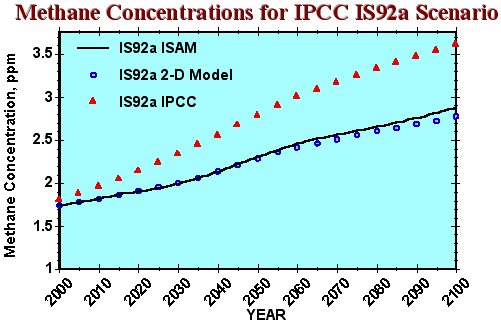 |
Figure 6: Click image to enlarge |
In Figure 6 the ISAM CH4 concentration results for the IPCC IS92a scenario are compared with those of the 2-D model. Input to the 2-D model are zonal patterns of 1990 CH4, NOx, CO and NMHC emissions which are scaled by global emission rates which grow according to the IS92a scenario. The ISAM model reproduces the globally averaged 2-D model result up until 2060, after which the ISAM CH4 concentration is slightly higher; the close reproduction is not surprising since OH production from the 2-D model result was used to calibrate OH production in ISAM. However, this analysis of the IS92a scenario gives a CH4 concentration which is about 20% lower than the IPCC (1995) projection of 3.6 ppmv in 2100, which prescribed constant NOx , CO and NMHC emissions at 1990 rates.
CFCs HCFCs and N2O
The past and future atmospheric N2O, CFC-11, -12, -113, -114, -115, HCFC-22, Halon-1301, CCl4, and CH3CCl3 concentrations are calculated by a simple mass balance model described by Bach and Jain (1995). In this model, the annual concentrations of specific gasses are determined by their initial concentrations, and emission and removal rates. The removal rates of these gasses are assumed to be inversely proportional to their atmospheric lifetimes.
CLIMATE-OCEAN MODEL
We use the globally averaged energy balance climate upwelling-diffusion ocean model similar to that used by IPCC (1990, 1996) to predict greenhouse gas-induced climate change. The model determines the atmospheric temperature and the temperature ocean as a function of depth from the ocean surface to the ocean floor. This climate ocean model contains a vertically integrated atmosphere box, a mixed-layer ocean box, an advective-diffusive deep ocean, and a thin slab representing land thermal inertia. In the deep ocean, heat is transported upwards with an advection velocity, and downwards by physical processes represented by a single, effective diffusion coefficient. Perturbations in the net radiative forcing from CO2 and other trace gases and aerosols are computed using formulae of Harvey et al. (1997), which were derived from detailed radiative transfer model.
In the climate -ocean model four quantities must be prescribed: (1) the temperature sensitivity of the climate system, ?T2x, defined as the equilibrium surface temperature increase for doubling of atmospheric CO2 concentration; (2) the vertically uniform upwelling velocity for the global ocean, w; (3) the verically uniform thermal diffusitivity; k; and the warming of the polar ocean relative to the warming of the non-polar ocean, p.
SEA LEVEL MODEL
The effects of global warming on sea level are determined by four processes: (i) thermal expansion of the ocean water (ii) melting of mountain glaciers, (iii) ablation of the Greenland Ice Sheet, and (iv) ablation or accumulation of the Antarctic Ice Sheet (notably the West Antarctic Ice Sheet). The sea level model described in Jain et al. (1994) uses the calculated transient temperature changes to estimate sea level changes due to thermal expansion and melting ice.
FIGURES
Figure 1 | Figure 2 | Figure 3 | Figure 4 | Figure 5 | Figure 6